Understanding the Lattice Structure of Metals
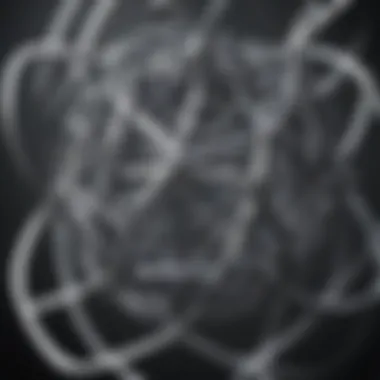
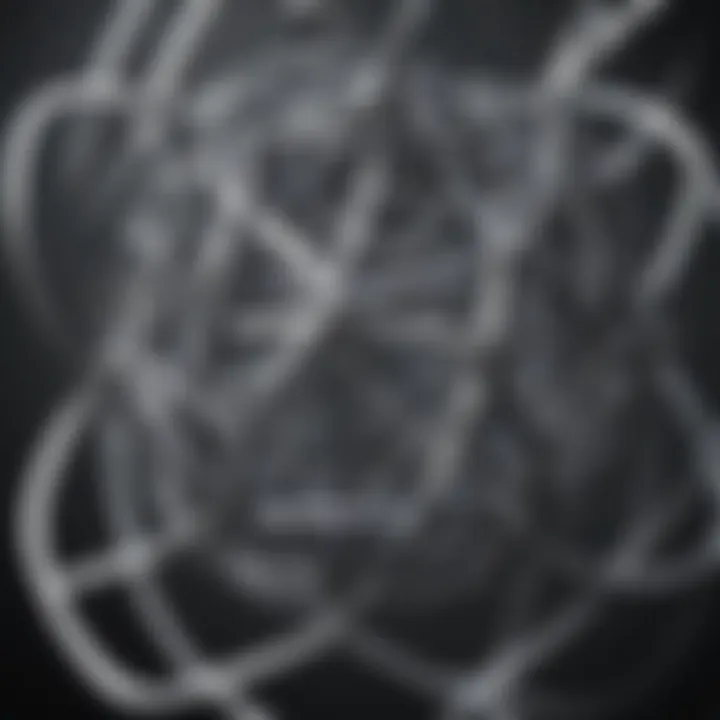
Intro
Metals play a pivotal role in modern society, forming the backbone of countless industries. At the heart of their vast array of properties lies an intricate system known as lattice structure. This invisible framework dictates not only how metals behave under various conditions but also their very suitability for specific applications.
Understanding the lattice structure of metals isn't merely an academic exercise; it's fundamental to the development of new materials and technologies. Whether it's the lightweight strength of titanium alloys in aerospace or the excellent conductivity of copper used in electrical systems, the arrangement of atoms within metallic lattices governs a multitude of characteristics.
In this article, we will embark on a detailed exploration of metallic lattice structures, considering their formation, types, and profound implications in material science. We aim to enhance comprehension among students, researchers, and professionals alike, providing substantial insights into why certain metals excel in specific roles while others do not.
Preamble to Lattice Structures
The lattice structure of metals is a cornerstone concept in materials science, underlining the very fabric of their properties. Understanding this arrangement goes beyond mere academic interest; it holds pivotal implications for various industries where performance and reliability are paramount. Every type of lattice arrangement provides unique characteristics, which affect how a metal behaves under stress, heat, or electricity. Therefore, comprehending these structures isn't just a theoretical exercise; it influences practical and technological advancements.
Lattice structures can be likened to the framework of a house. Just as the layout dictates the strength and stability of a building, the atomic arrangement in metals determines their mechanical and physical behavior. When we speak of lattice structures, we are diving into the organized chaos of how atoms bond, align, and interact on a microscopic scale. This understanding aids in predicting how metals can be utilized effectively in different applications.
The importance of knowing lattice structures comes also from the continuous pursuit of innovation in material science. As industries evolve, there is an ever-growing need for materials that not only meet but exceed performance expectations. Researchers and engineers must recognize how the aligning of atoms affects corrosion resistance, thermal behavior, and even weight reduction in advanced applications.
"The arrangement of atoms is not just a matter of structure; it is the essence of function and versatility in material applications."
Thus, introducing the concept of lattice structures right from the get-go sets the stage for a deeper exploration into the specifics of metal behavior. By laying out the framework of skills and knowledge regarding lattice structures, we create a platform for further discussions about their types, formation, and impact on material properties.
Defining Lattice Structure
A lattice structure can be succinctly described as a systematic arrangement of atoms in a solid. This arrangement is characterized by its symmetry and repetition, forming a three-dimensional grid. Each point in this grid corresponds to an atom, and the geometry of this grid dictates how different kinds of atoms interact with each other. There are various types of lattice structures, each with a unique configuration: cubic, hexagonal, and more.
When delving into lattice structure, one important consideration is the concept of unit cells. A unit cell is the smallest repeating unit of a lattice that encapsulates its symmetry and forms the foundation for the entire structure. In metals, these unit cells can exist in varying shapes, leading to different properties such as stability and reactivity.
Historical Context
Historically, the study of lattice structures can be traced back to the early 20th century, when scientists began employing X-ray diffraction techniques to ascertain atomic arrangements. Pioneers like William Lawrence Bragg and his father, William Henry Bragg, contributed significantly to the field of crystallography. Their work laid the foundation for understanding the periodic nature of atoms in metallic lattices.
This deepened our insight into how metals like iron, copper, and aluminum exhibit distinct physical characteristics based on their lattice types. For instance, the discovery of the face-centered cubic structure revealed why some metals are malleable, while others display superior strength. Over the decades, advancements in technology have only expanded our ability to analyze these structures, leading to breakthroughs that affect everyday products and advanced technology alike.
Understanding the historical background adds depth to our appreciation of lattice structures, demonstrating that the discussion is rooted not only in theory, but also in tangible advancements in material science. It highlights the ongoing journey from fundamental research to practical applications, urging us to continue exploring this fascinating topic.
Types of Lattice Structures in Metals
Understanding the variety of lattice structures in metals is crucial for anyone diving into the realm of material science. These structures dictate a wide range of properties and behaviors, from mechanical strength to thermal conductivity, making this knowledge indispensable for engineers, researchers, and educators alike. By examining different lattice types—Body-Centered Cubic (BCC), Face-Centered Cubic (FCC), and Hexagonal Close-Packed (HCP)—one can gain insight into the underlying reasons why certain metals are favored in specific applications.
Body-Centered Cubic (BCC)
Characteristics of BCC
The BCC lattice is distinguished by its unique arrangement of atoms, where one atom occupies the center of a cube formed by eight other atoms at the corners. A key characteristic of this structure is its lower atomic packing efficiency, around 68%, leading to a distinctive distribution of interstitial spaces. This setup contributes significantly to certain mechanical properties, such as higher yield strength and moderate ductility, making BCC metals particularly versatile in applications requiring robustness.
Moreover, BCC structures have an intriguing behavior under stress. When subjected to force, their atoms can shift in such a way that prevents premature failure. This is beneficial for tools and machinery that must endure rigorous conditions. However, it’s worth noting that BCC metals often exhibit less toughness at lower temperatures, which can be a limitation in colder environments.
Examples of BCC Metals
Some prime examples of BCC metals include iron, chromium, and tungsten. Iron, for instance, exhibits a transformation to BCC when cooled to lower temperatures, which adds to its structural integrity in construction. Chromium complements this with its resistance to corrosion, and tungsten is revered for its high melting point, making it a preferred option for high-temperature applications.
The BCC structure’s ability to withstand various temperatures and loads positions these metals as essential components in many engineering applications, although their relatively brittle nature when cold needs to be considered.
Face-Centered Cubic (FCC)
Characteristics of FCC
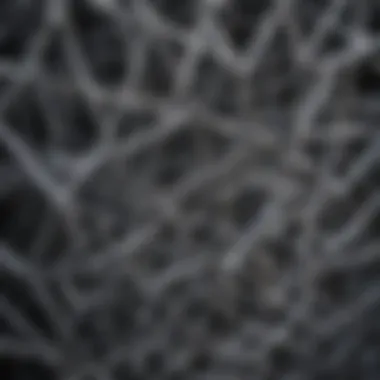
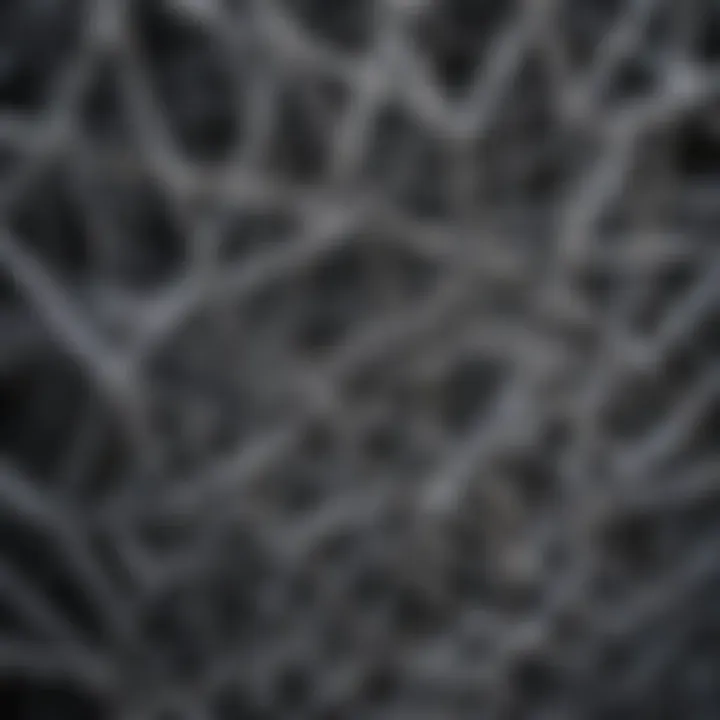
FCC structures are characterized by a more compact arrangement, where atoms occupy the corners and the centers of each face of the cube. This configuration yields a higher packing efficiency of about 74%, resulting in enhanced ductility and toughness. One of the standout features of FCC metals is their resistance to deformation at room temperature, which leads to superior workability.
This malleability stems from the positioning of slip planes—structures along which deformation can occur. The multiple slip systems in FCC allow for easier movement of dislocations, granting metals such as aluminum and copper a flexibility that makes them suitable for various processing techniques, from rolling to forging.
Examples of FCC Metals
Aluminum, copper, and gold are some prominent examples of FCC metals. Aluminum shines in structural applications thanks to its light weight and resistance to corrosion. Copper, known for its excellent electrical conductivity, is widely used in electrical wiring. Gold, while often viewed as a precious material, also finds utility in electronics, owing to its superb conductivity and resistance to oxidation.
Importantly, FCC metals' ability to maintain toughness under stress makes them particularly beneficial in industries that demand reliability, such as aerospace and automotive manufacturing.
Hexagonal Close-Packed (HCP)
Characteristics of HCP
HCP structures present a different geometric arrangement, where atoms are densely packed in hexagonal layers. The coordination number in HCP is 12, which permits a remarkable packing efficiency of about 74%, akin to FCC. However, the difference lies in the slip systems available for deformation. Because HCP metals have fewer slip systems compared to FCC structures, they often exhibit limited ductility at room temperature.
Despite this limitation, HCP metals can endure significant stress before failing, making them incredibly valuable in demanding applications. Additionally, their high strength-to-weight ratio is an advantage that shouldn't be overlooked.
Examples of HCP Metals
Common HCP metals include magnesium, titanium, and zinc. Magnesium is utilized in lightweight applications, particularly in the automotive sector, where fuel efficiency is critical. Titanium, on the other hand, is lauded for its exceptional strength-to-weight ratio and corrosion resistance, ideal for aerospace components. Zinc often plays a role as a coating to protect steel, leveraging its properties to prevent rust and extend the life of structures.
Each of these HCP metals showcases unique advantages that make them vital in engineering, yet their limited ductility must be accounted for in designs.
Formation of Lattice Structures
The formation of lattice structures is a foundational aspect of metallurgy and material science. It's more than just the arrangement of atoms; it lays the groundwork for understanding how metals behave under various conditions. This not only affects the material's physical properties but also impacts how they can be applied in real-world situations. The processes governing the formation of these structures are intricate, revealing the delicate balance between nature and mechanics.
Nucleation and Growth Mechanisms
Nucleation marks the initial formation of a lattice. This is a critical stage, as it represents the point where atoms begin to arrange themselves in a structured form. There are primarily two types of nucleation: homogeneous and heterogeneous. In homogeneous nucleation, new phases form uniformly throughout the material, while heterogeneous nucleation occurs at surfaces or defects. This latter is common in most practical applications, as impurities and surface irregularities often provide a favorable environment for nucleation to take hold.
The growth mechanisms of lattice structures are equally important. Once nucleation occurs, the growth phase determines how the lattice expands and evolves. There are several growth mechanisms including diffusion, where atoms migrate to the growing phase; and interface-controlled growth, which involves the movement of the boundary between different phases. Understanding these mechanisms allows researchers to manipulate and optimize metal properties deliberately.
Factors Influencing Lattice Structure Formation
Numerous factors affect how lattice structures form, with temperature and pressure being two of the primary influences.
Temperature Effects
Temperature plays a crucial role in lattice structure formation. It directly impacts atomic mobility, which is the ability of atoms to move within a material. At higher temperatures, atoms gain energy, making it easier for them to move into positions that contribute to a stable lattice structure. This is why many metal forming processes, like casting or forging, are performed at elevated temperatures.
One key characteristic of temperature effects is the adjustment of phase transitions. For example, iron transitions from body-centered cubic to face-centered cubic at around 912 degrees Celsius. This transition can dramatically change the mechanical properties of the metal, making it more ductile or brittle depending on the temperature employed during processing.
Always keep in mind, though, that high temperatures can introduce unique challenges, like grain growth which can affect the uniformity of the lattice and lead to weak points in the material.
Pressure Conditions
Pressure conditions also play a pivotal role in lattice structure formation. Applying pressure can enhance the packing efficiency of atoms, allowing for a denser lattice arrangement. This is particularly relevant in processes like sintering, where increased pressure can lead to improved mechanical properties through better atom contact and bond formation.
One important aspect of pressure effects is how they enable phase transformations. For instance, carbon can transition from graphite to diamond under high pressure, resulting in a lattice that is not only different in arrangement but also vastly superior in strength.
Nevertheless, the adverse side of high pressure can result in unwanted defects or even structural failure if not managed correctly. Careful control of those parameters is vital for maintaining the desired lattice quality.
"A deep understanding of how temperature and pressure influence the formation of lattice structures helps in tailoring materials for specific applications. This knowledge is essential for researchers and engineers alike."
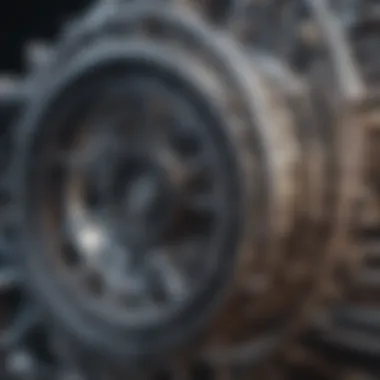
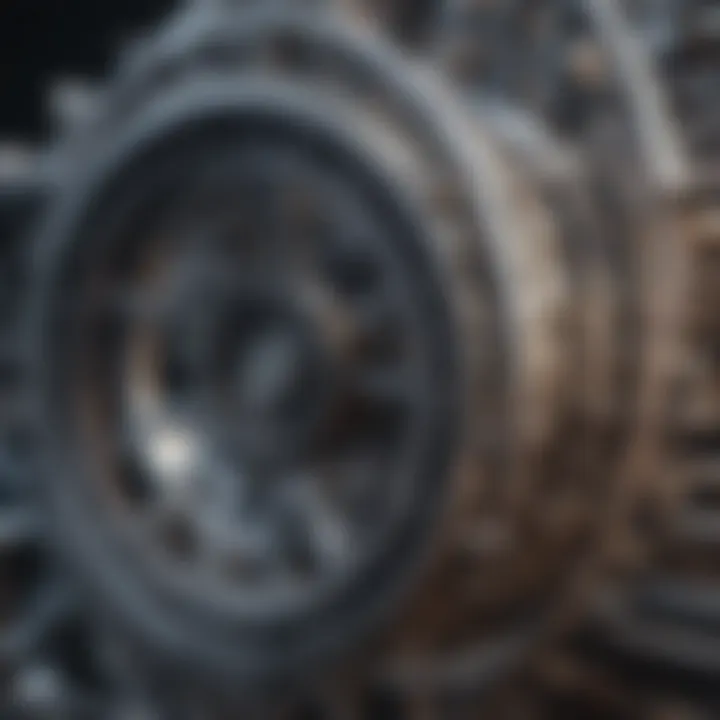
In sum, both temperature and pressure are key considerations when discussing the formation of lattice structures in metals. By examining these factors in depth, we uncover significant insights into how metals can be engineered to meet specific functional requirements.
The Role of Lattice Structures in Material Properties
The arrangement of atoms within a metal's lattice structure profoundly impacts its physical and chemical properties. Understanding this relationship is crucial to various applications across sectors such as aerospace, construction, and electronics. Material properties—including the mechanical strength, ductility, thermal management, and electrical conduction of metals—are directly tied to their respective lattice configurations. This section will unwrap how these lattices not only dictate the behavior of metals under stress but also influence their utility in technology and engineering.
Mechanical Properties
Strength and Ductility
Strength and ductility are two sides of the same coin when discussing the characteristics of metals. Strength typically refers to a material's ability to withstand an applied force without failure, while ductility indicates how much deformation a material can undergo before breaking. In simple terms, a strong material can take a beating, while a ductile one can bend without snapping.
The crystal lattice arrangement plays a pivotal role here. For instance, metals with a face-centered cubic (FCC) structure, such as aluminum and copper, exhibit excellent ductility alongside moderate strength. This unique combination allows these metals to be shaped and formed through processes such as drawing and rolling while maintaining integrity. On the other hand, body-centered cubic (BCC) metals like iron tend to favor strength over ductility, making them a solid choice for structural applications but less ideal where extensive shaping is necessary.
One unique feature of the interplay between strength and ductility is the concept of the yield point, which denotes the stress level at which a material begins to deform permanently. In FCC metals, the yield point is higher, allowing for more significant deformation before failure. However, the challenge lies in the trade-off: if you push for greater strength in alloys, you may lose ductility, which can lead to brittleness. This complexity highlights the careful balancing act design engineers face when selecting materials for specific applications.
Toughness and Hardness
When diving deeper into mechanical properties, we encounter toughness and hardness. Toughness describes a metal's ability to absorb energy and withstand fracturing, while hardness denotes resistance to deformation. Together, they paint a fuller picture of how a metal will perform under mechanical stress.
Toughness is greatly influenced by the lattice structure. Typically, a lattice that allows for more slip systems, like that in FCC metals, results in higher toughness. Tough metals can absorb impacts that would shatter harder, less ductile materials. A prime example is the toughness of steel alloys, which amalgamate both hardening features and a ductile base to prevent catastrophic failure.
On the opposite side, hardness is often increased through treatments like heat or surface hardening, yet this can often compromise toughness. Take hardened steel, known for its surface hardness—ideal for cutting tools—yet prone to brittleness when subjected to shock loads. Hence, when designing components intended for high shock applications, engineers must be wary of these trade-offs to avoid catastrophic failures.
Thermal and Electrical Conductivity
Influence of Lattice Structure on Conductivity
Metals are revered for their excellent thermal and electrical conductivity, which fundamentally hinges on their lattice structure. The ease with which electrons flow through the lattice significantly affects both thermal and electrical properties. For example, copper, which possesses a very efficient FCC structure, excels in both categories. Its structure permits the swift movement of free electrons, allowing it to effectively conduct heat and electricity.
Moreover, lattice defects like vacancies can lead to altered conductivity levels. When these imperfections exist, they can impede electron flow, increasing resistivity. Meanwhile, silver stands out as the best electrical conductor; its unique metallic bonding and face-centered cubic structure allow for even greater electron mobility than copper.
Comparative Analysis of Conductivities
When evaluating various metals and their conductivities, significant variations arise due to their unique lattice arrangements. For instance, comparing copper and aluminum reveals copper's superior conductivity, attributable to its tighter atomic packing and enhanced electron flow pathways.
- Key Characteristics of Conductivities by Element:
- Copper: Excellent conductivity due to FCC structure, widely used in electrical wiring.
- Aluminum: Good conductivity but less than copper; however, its lightweight properties make it a preferred choice in aerospace.
- Gold: Offers remarkable conductivity and corrosion resistance but at a higher cost.
The comparative analysis points to the importance of lattice configuration in determining not just performance but also suitability for specific applications. This knowledge helps materials scientists tailor solutions to needs, ensuring that materials employed not only meet but exceed performance criteria in their intended use.
Applications of Lattice Structures
Understanding lattice structures in metals goes beyond mere academic interest; it fuels the very heart of industrial innovation and technological progress. The unique arrangements of atoms in these metallic lattices play a crucial role in determining the properties of materials, which in turn, influences their applications across various sectors. The significance of lattice structures can be observed in diverse fields such as aerospace engineering and construction materials, as well as in the rapidly evolving landscape of technology, particularly in semiconductors and nanotechnology. As we delve into these applications, we uncover both the strengths and limitations of different lattice forms, shedding light on why certain metals are chosen for specific roles over others.
Industrial Applications
Aerospace Engineering
Aerospace engineering is a field where the selection of materials is critical, and the lattice structure of metals can make or break a design. The key characteristic of materials used in this field is the need for high strength-to-weight ratios. Metals with body-centered cubic configurations, such as titanium alloys, illustrate this perfectly. Their unique atomic arrangement leads to exceptional strength without excessive weight, making them ideal for aircraft components.
A major advantage of using metals with strong lattice structures in aerospace is reduced fuel consumption. Lighter materials reduce the energy required for flight, leading to more efficient engines and lower emissions. However, there are also disadvantages; for instance, the cost of high-performance alloys can be substantial, which sometimes limits their application to only high-end or military vehicles. Overall, the link between lattice structure and performance emphasizes the necessity of a deep understanding of material properties for designing safe and effective aerospace solutions.
Construction Materials
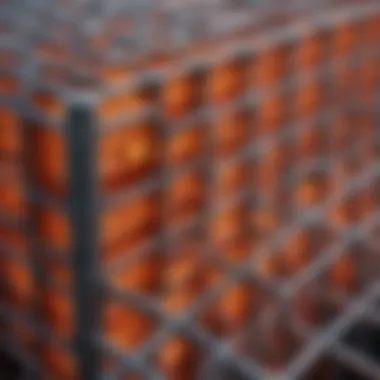
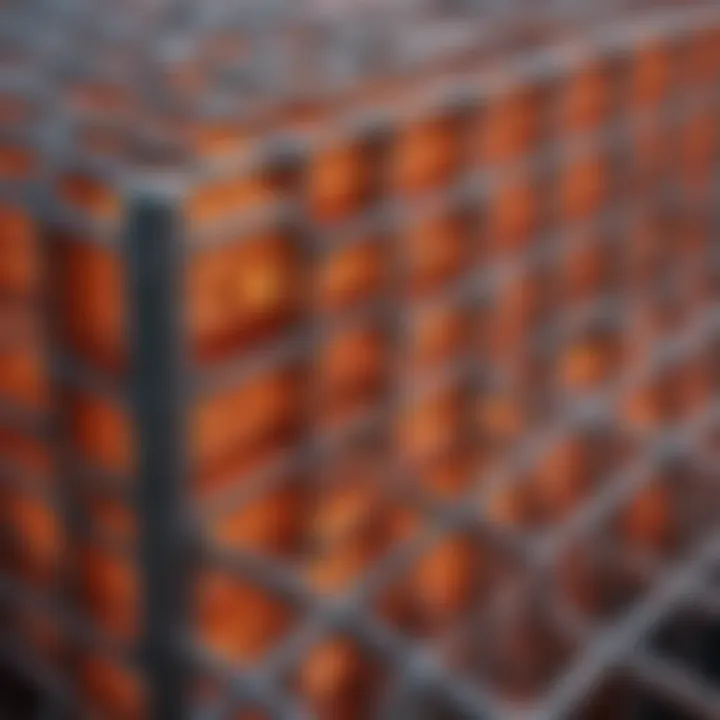
In construction, the importance of lattice structures is equally paramount. Steel, with its face-centered cubic lattice, offers remarkable tensile strength and resilience. This beneficial quality allows it to support enormous weight, making it a go-to material for skyscrapers and bridges. The unique feature of steel is that it can be alloyed with other elements, enhancing its properties while maintaining cost-effectiveness, unlike pure metals that might not provide that flexibility.
However, while sturdy, steel has its disadvantages; it can be susceptible to corrosion over time, necessitating protective coatings or regular maintenance. Consequently, understanding how lattice structures impact durability and longevity is vital when selecting materials for construction. Balancing these attributes against costs and maintenance requirements becomes a key consideration.
Technological Advances
Semiconductors
Semiconductors represent a realm where lattice structures are essential for functionality. Elements like silicon possess a diamond cubic lattice that allows for precise control of electrical properties, making them a desirable choice for electronic applications. The specific aspect of semiconductors is their ability to conduct electricity under certain conditions while acting as insulators under others, a feature that's rooted in their specific crystalline arrangement.
The inherent advantage of using silicon in technology lies in its compatibility with existing manufacturing processes, which has driven its widespread adoption. Nevertheless, as the demand for smaller and more efficient devices grows, researchers are continuously exploring materials with alternative lattice structures to push the boundaries of what semiconductors can achieve.
Nanotechnology
In the field of nanotechnology, the role of lattice structures takes on a new dimension. Materials are engineered at the atomic level, and the arrangements of these atoms dictate their unique properties and behaviors. One of the key characteristics of nanotechnology is the ability to manipulate materials to enhance their performance, leading to applications such as drug delivery systems and advanced computing.
The unique feature of nanomaterials is their increased surface area-to-volume ratio, which allows for more efficient chemical reactions, making them highly effective in catalysis and other applications. However, challenges also exist, such as the complications in synthesizing nanomaterials consistently and the potential environmental impacts. Thus, as we advance in this field, a careful consideration of lattice structures helps guide research and application towards more sustainable solutions.
Challenges in Understanding Lattice Structures
Understanding lattice structures in metals is no walk in the park. While this field is packed with valuable insights, it’s also riddled with complexities that often leave researchers scratching their heads. Grasping the intricate details of how these structures form and behave is paramount, not just for theoretical knowledge but also for practical applications in material science.
Limitations of Current Research
In the current landscape, researchers face a multitude of barriers when delving into lattice structures. One significant limitation is the reliance on models that might oversimplify the real-world conditions. Many existing studies lean heavily on computational simulations that do not always account for diverse environmental factors like temperature and pressure variations.
- Computer Models: These models, while useful, sometimes fail to replicate the complexities of real-life scenarios. As a result, the data acquired can be skewed or inaccurate, leading to a misunderstanding of how materials behave under actual conditions.
- Material Variability: Another stumbling block is the inherent variability of materials themselves. Not all samples of a metal will have the same lattice structure, hence drawing universal conclusions can be misleading.
Overcoming these limitations requires not only improved models but a multifaceted approach that merges theory with empirical data. Understanding that progress in this field often runs into a wall of limitations can provide a more realistic outlook and prompt the need for a more thorough and diverse inquiry.
Future Research Directions
Given the limitations outlined above, it's crucial to explore future avenues of research in lattice structures. These directions could pave the way for substantial advancements and new understandings.
Advanced Computational Models
One promising area of exploration revolves around advanced computational models. Such models are increasingly becoming sophisticated, allowing researchers to analyze complex interactions within lattice structures.
- Key Characteristic: The principal feature of these advanced models is their ability to integrate real-world variables into their simulations. They can account for varying temperatures, pressure changes, and even atomic-level interactions that were often overlooked in earlier models.
- Benefits: This results in a more nuanced and accurate portrayal of how metals behave, bridging the gap between theoretical predictions and practical realities.
- Unique Features: These models often come equipped with machine learning algorithms, making them adaptable and capable of producing predictive insights based on incomplete data.
In this context, their contributions to the overall understanding of lattice structures are invaluable. They offer a broader perspective and help refine existing theories.
Experimental Techniques
In parallel with computational advancements, there is a pressing need to harness innovative experimental techniques. These methodologies can complement theoretical models and reveal insights that simulations alone cannot provide.
- Key Characteristic: A notable feature of these experimental settings is their ability to manipulate the environment precisely, thus allowing for controlled studies. By simulating different conditions, researchers can directly observe how lattice structures respond.
- Benefits: The results from such experiments often yield concrete data that can validate or challenge existing theoretical frameworks. Having tangible data means improving the accuracy of computational models as well.
- Unique Features: Techniques like X-ray diffraction and neutron scattering provide high-resolution insights into the atomic arrangements, enabling deeper dives into the characteristics of lattice structures.
Ending
In this examination of metallic lattice structures, we have uncovered pivotal insights into their role within material science and metallurgy. Concluding this journey, it becomes evident that the arrangement of atoms within metallic lattices is not merely a subject of academic interest; it bears substantial implications on a plethora of practical applications. Understanding these structures is crucial for both researchers and industry professionals striving to innovate in materials design.
Summary of Key Insights
- Lattice Structures Influence Properties: Various lattice arrangements—BCC, FCC, and HCP—play a definitive role in determining mechanical properties such as strength and ductility. The alignments of these atoms contribute to how metals respond under stress, heat, or electrical flow.
- Applications Span Multiple Industries: With unique characteristics arising from their lattice formations, metals find their places in a wide range of applications. From construction materials in building durable bridges to specialized alloys in aerospace applications, the lattice structure governs suitability.
- Future Directions in Research: There is an ongoing need for advancement in computational models and experimental techniques to further untangle complexities associated with different lattice structures. Embracing these facets can lead to the development of groundbreaking materials that outperform current standards.
Understanding these insights not only enriches the academic perspective but also fosters informed decisions in practical applications, bridging the gap between theory and functionality.
The Importance of Lattice Structure Study
The study of lattice structures transcends the boundaries of theoretical physics and materials science, delving into a fundamental aspect of how we utilize metals in our daily lives. As we navigate an increasingly technological world, materials that can perform under extreme conditions are in ever-growing demand. Lattice structures directly impact thermal and electrical conductivities, and understanding these relationships becomes crucial in industries such as electronics and energy.
Moreover, as artificial intelligence and automation become more prevalent, a nuanced grasp of material behavior will underpin innovations in manufacturing processes and product development. A careful study of lattice phenomena enables designers to predict how new materials behave, ultimately leading to better performance and greater efficiency. The significance of studying lattice structures also lies in its ability to prepare researchers and engineers to tackle challenges posed by emerging technologies.