Exploring the Fundamentals of X-ray Diffraction
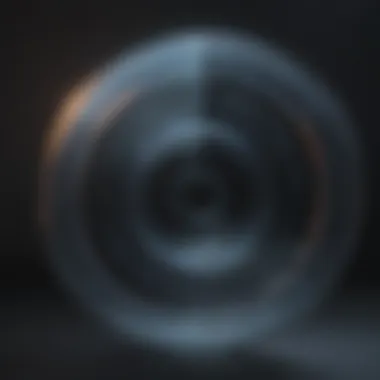
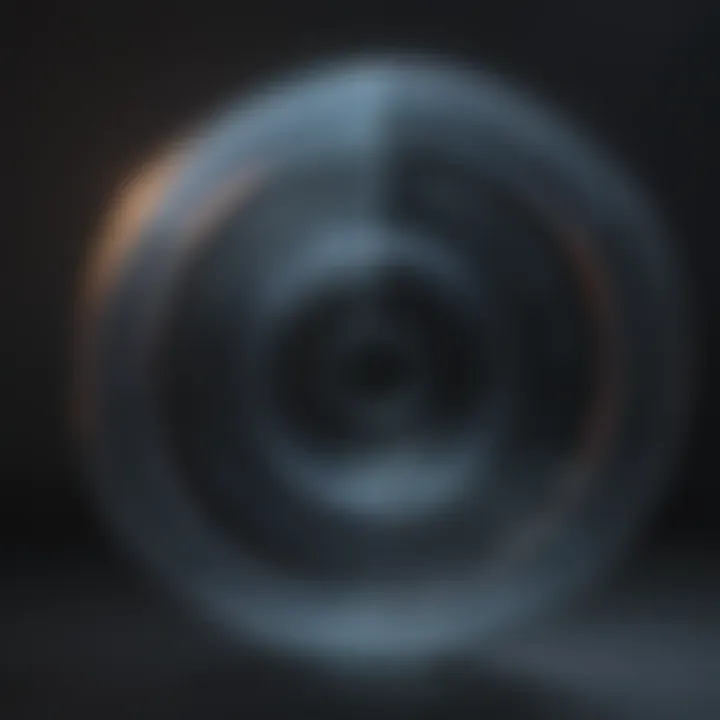
Intro
X-ray diffraction (XRD) stands as a cornerstone in the analytical toolkit of scientists exploring the intricate world of materials at the atomic level. At its core, this technique deciphers the arrangement of atoms within crystalline substances by examining the patterns created when X-rays scatter off the material. Its applications span across various scientific domains, from materials science to chemistry and biology, making it an integral part of research and development.
The beauty of X-ray diffraction lies not just in its findings but also in its methodologies and constant evolution. Understanding the principles behind XRD allows researchers and practitioners to leverage its potential effectively. This article endeavors to unpack the complexities of XRD, shine a light on its crucial advancements, and illustrate its pivotal role in various scientific inquiries. Through a thorough investigation, we aim to provide valuable insights that will enhance comprehension among students, educators, and professionals alike.
In this immersive journey, we will explore not only the foundational concepts but also the latest technological enhancements in XRD. Our aim is to establish a firm understanding of how XRD facilitates innovations, ultimately aiding in finding solutions to pressing challenges across numerous fields.
Thus, let’s commence with the research overview, laying the groundwork for our exploration into the fascinating realm of X-ray diffraction.
Preface to X-ray Diffraction
X-ray diffraction, often referred to as XRD, holds a central place in modern scientific research. Its power lies in its ability to unveil the arrangement of atoms within a material. Understanding x-ray diffraction not only illuminates the structure of various substances but also enhances our capability to design and develop materials with tailored properties. From mapping out crystal structures to studying complex biological molecules, XRD has broad implications across diverse fields, including materials science, chemistry, and biology.
Definition and Importance
X-ray diffraction is a technique used to determine the atomic and molecular structure of a crystal. When a beam of x-rays hits a crystalline sample, it scatters in different directions, yielding a diffraction pattern. This pattern provides critical insights into the internal organization of matter. Here are a few key points about its importance:
- Structural Determination: It provides precise information regarding the unit cell dimensions and symmetry of the crystalline structure.
- Material Characterization: XRD can be instrumental in analyzing the purity and phase of materials, which can be crucial for quality control in manufacturing.
- Research Applications: It's extensively used in various research domains including solid-state physics, chemistry, and materials science to uncover the properties of new compounds.
In essence, the relevance of x-ray diffraction is multifaceted. It serves as a bridge between theory and practical applications, offering insight into a universe that is otherwise hidden from sight.
Historical Context
The groundwork for x-ray diffraction was laid in the early 20th century. It all began shortly after the discovery of x-rays by Wilhelm Röntgen in 1895. But it was Max von Laue who, in 1912, demonstrated the diffraction of x-rays by crystals. This groundbreaking experiment was not merely a twist in scientific curiosity; it revolutionized how scientists could view the atomic world.
Carl Friedrich Bragg, along with his son William Henry Bragg, later formalized the theoretical underpinnings of x-ray diffraction. Their work led to the formulation of Bragg's Law, which quantitatively describes the angle of diffraction, based on the wavelength of the x-ray and the distance between planes in the crystal.
Since its inception, x-ray diffraction has been at the forefront of crystallography, allowing researchers to progress from determining simple structures to deciphering complex biomolecules, such as proteins and nucleic acids, thereby impacting multiple scientific fields profoundly. The historical evolution of this technique showcases not only its scientific value but also its role in advancing human knowledge.
Fundamental Principles of X-ray Diffraction
Understanding the fundamental principles of X-ray diffraction (XRD) is crucial for grasping how this technique operates and why it holds significant value in material characterization and structural analysis. XRD essentially hinges on the wave nature of X-rays and the periodic arrangement of atoms within a crystal. The synergy of these aspects leads to constructive and destructive interference patterns that ultimately yield information about the crystal structure.
In this section, we will explore the theoretical framework of XRD, including Bragg's Law and the reciprocal lattice concept. Moreover, the nature of X-rays will be examined, highlighting their production and interaction with matter. This exploration not only lays a foundation for practical applications but also sharpens the lens through which researchers view material properties and behaviors.
Theoretical Framework
Bragg's Law
Bragg's Law is the cornerstone of X-ray diffraction, defining the conditions under which constructive interference occurs inside a crystal lattice. The law can be succinctly expressed with the equation:
[ n\lambda = 2d\sin(\theta) ]
In this equation:
- ( n ) is the order of the reflected beam,
- ( \lambda ) is the wavelength of the incident X-rays,
- ( d ) is the distance between the crystal planes,
- ( \theta ) is the angle of incidence.
The beauty of Bragg's Law lies in its simplicity; it ties the geometric spacing of crystal planes to the wavelengths of X-rays. This leads to a clear understanding of how various materials can exhibit specific diffraction patterns based on their crystal structures. The practical implication of this law makes it an exceptionally popular choice for both novice and expert researchers.
One notable characteristic of Bragg's Law is its straightforward application in identifying the orientation of crystals. It is especially compelling because of its ability to provide insight into crystallographic parameters without requiring advanced computational methods.
Advantages and Limitations:
- Advantages:
- Limitations:
- Provides direct relationship between X-ray wavelength and crystal structure.
- Facilitates easy interpretation of data obtained from diffraction patterns.
- Assumes a perfectly ordered crystal, which may not be the case in real-world materials.
- Limited in describing complex structures or non-crystalline materials.
Reciprocal Lattice Concept
The reciprocal lattice is a powerful conceptual tool in crystallography that aids in visualizing the diffraction patterns of crystalline materials. Unlike real space, which describes the physical arrangement of atoms, the reciprocal lattice represents the Fourier transform of the crystal structure. Each point in this lattice corresponds to a possible diffraction peak observable in an XRD pattern.
One of the key characteristics of the reciprocal lattice is its ability to simplify the understanding of diffraction processes. It elegantly shows how various crystal orientations and spacings relate to the angles and intensities of diffracted rays, allowing researchers to predict and interpret diffraction patterns more accurately.
The unique feature of the reciprocal lattice is its three-dimensional nature, which enables the analysis of complex diffraction patterns that arise due to varied crystallographic orientations. This is immensely beneficial for studies that involve multi-phase materials or intricate crystal structures.
Advantages and Limitations:
- Advantages:
- Limitations:
- Provides a clear framework for visualizing and interpreting diffraction data.
- Simplifies calculations related to diffraction conditions based on crystal symmetry.
- Requires a solid understanding of mathematical concepts associated with Fourier transforms.
- Can become complex when dealing with non-standard crystal geometries.
Nature of X-rays
Production of X-rays
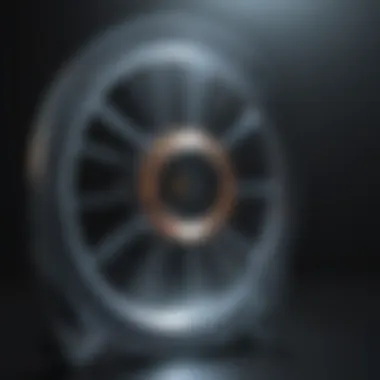
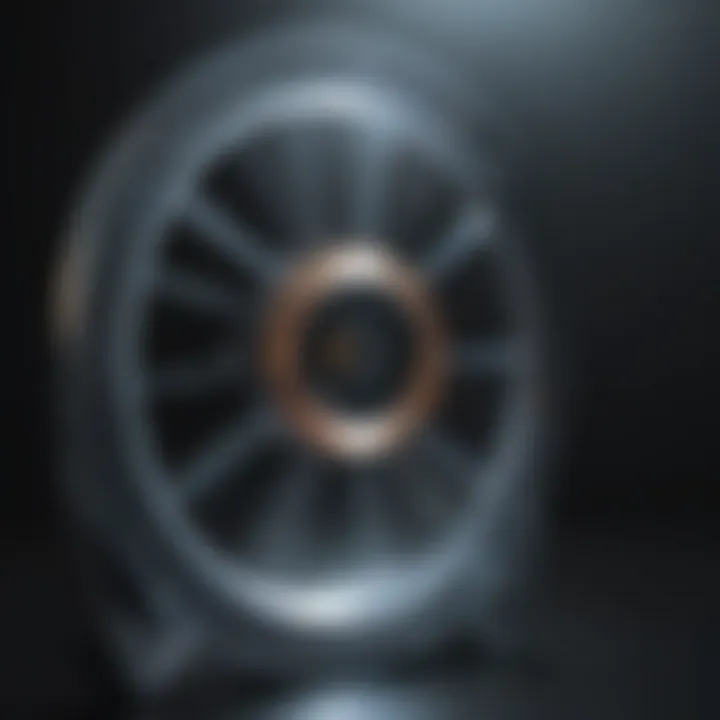
The generation of X-rays primarily involves two methods: characteristic radiation and Bremsstrahlung. In the characteristic method, high-energy electrons collide with a metal target, exciting the inner-shell electrons and causing them to emit X-rays as they return to their stable state. Conversely, Bremsstrahlung occurs when high-speed electrons are decelerated upon interaction with the target’s nuclei, emitting X-rays in the process.
Understanding the production of X-rays is vital for comprehending how variations in energy levels result in different wavelengths, impacting the resolution and penetration power of the X-rays in diffraction studies.
One key characteristic of X-ray production is that commercially available X-ray sources can produce a range of wavelengths, allowing scientists to tailor their experiments. This adaptability is a significant benefit in XRD, making it easier to study various materials across different fields.
Advantages and Limitations:
- Advantages:
- Limitations:
- Provides flexibility for researchers to choose appropriate wavelengths for specific studies.
- Adaptable to various experimental requirements, enhancing the technique's versatility.
- The quality of X-rays generated can vary with equipment and settings, influencing results.
- Not all materials are suitable for X-ray analysis due to absorption or scattering effects.
Interaction with Matter
The interaction of X-rays with matter is a fundamental aspect that determines the effectiveness of XRD. When X-rays encounter a material, they can be absorbed, scattered, or transmitted, with diffraction being a specific scattered outcome that preserves information about the crystal structure.
A distinctive characteristic of X-ray interaction is the elastic scattering that occurs predominantly with electrons in atoms. This is relevant because it enables researchers to ascertain structural information without significantly altering the material being analyzed.
Advantages and Limitations:
- Advantages:
- Limitations:
- Non-destructive to samples, preserving material integrity during analysis.
- Offers rich structural insight based on how X-rays interact with different atomic arrangements.
- The technique can be less effective with amorphous materials, leading to ambiguous data.
- Elements with high atomic numbers may absorb X-rays more efficiently, complicating analysis.
Technical Aspects of XRD
The discussion of technical aspects in X-ray diffraction is not just a stroll down memory lane but a crucial part of grasping how effective and efficient this technique is in today’s research landscape. Understanding the right instrumentation and the nuances of sample preparation can amplify the quality and reliability of results obtained from XRD experiments. This section illuminates essential elements of instrumentation and sample preparation techniques that are instrumental in carrying out successful XRD analyses.
Instrumentation
The realm of instrumentation in X-ray diffraction is a fascinating territory, filled with various components that each play a key role in generating reliable data. The right instruments can vastly influence measurement fidelity, giving researchers the tools they need to unearth valuable insights into crystallographic structures.
X-ray Sources
X-ray sources serve as the backbone of an XRD setup. Various types exist, each with its unique advantages. In general, laboratory-based X-ray tubes provide a consistent source of X-rays, allowing for controlled conditions during experiments. One notable characteristic of these sources is their ability to produce continuous spectra, which is essential for obtaining high-quality diffraction patterns.
A beneficial aspect of X-ray tubes is their relatively compact size, making them suitable for academic laboratories and smaller research facilities. However, a potential setback is that they may not reach the higher energies required for certain applications, limiting the range of studies that can be performed. On the other hand, synchrotron radiation offers an incredibly intense and collimated beam, empowering researchers to analyze materials at a level of detail that standard lab sources simply can’t match.
The downside, of course, is the accessibility, as synchrotron facilities are not available to everyone and often require long-term planning to acquire beam time.
Detectors and Data Collection
When diving into the depths of any XRD investigation, how data is collected matters significantly. Detectors convert the X-ray signals into usable data that reveals the structure of the materials under scrutiny. Position-sensitive detectors (PSDs) are a highlight in this area due to their ability to provide rapid detection across a wider angle range. This means researchers can gather more information in less time, boosting efficiency.
However, these detectors can sometimes bring along complexity, as analyzing the data extracted can require more sophisticated software and associated training. Contrastingly, traditional scintillation counters are simpler and can offer ease of use. Yet, they often lack the speed needed for high-throughput applications.
Sample Preparation Techniques
Proper sample preparation stands as a fundamental pillar in the XRD process, as it can heavily influence the quality of the data collected. The methods selected depend largely on the nature of the materials being analyzed, making this section pivotal in ensuring concise and accurate XRD results.
Powder Diffraction
Powder diffraction is one of the most common methods utilized in XRD, especially suitable for polycrystalline materials. This technique works on the principle that random orientations of crystalline grains will lead to unique diffraction patterns, giving a robust overview of structural information. An attractive feature of powder diffraction is that it’s generally easier and more straightforward to prepare samples compared to single crystals, allowing researchers to analyze a wider variety of materials.
However, the downside can be the potential loss of structural information, as the averaging of multiple orientations might obscure features that would be evident in single crystal analysis.
Single Crystal Methods
On the other end of the spectrum is single crystal XRD, which allows for the examination of the structure of a material in exquisite detail. This technique requires the meticulous and often time-consuming process of growing high-quality crystals. The key advantage here is an abundance of crystal data—each measurement can reveal intricate details that cannot be seen with the powder diffraction approach.
Nevertheless, single crystal methods come with significant limitations—it’s not always straightforward to produce suitable crystals, and the effort spent may not always yield the desired results. Additionally, interpreting the detailed data can demand a greater level of skill and experience, further complicating its application.
"In X-ray diffraction, the choice of technique—be it powder or single crystal—can significantly shape the adventure of materials characterization."
Ultimately, understanding these technical aspects of XRD enhances the ability to tackle various scientific questions, which in turn can accelerate discoveries across multiple fields.
Applications of X-ray Diffraction
X-ray diffraction (XRD) serves as a cornerstone technique in numerous scientific fields by allowing researchers to analyze the atomic structure of materials. This section delves into the various applications of XRD, emphasizing its significance in materials science, chemistry, and biological research. The ability to garner detailed insights about crystalline structures not only contributes to fundamental science but also finds pivotal roles in practical applications across industries. The versatility of XRD as a tool for characterizing materials makes it invaluable in both academic and industrial settings.
Materials Science
Analysis of Crystalline Materials
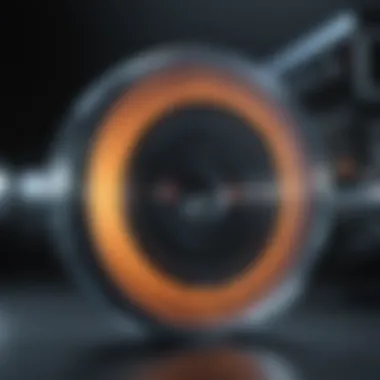
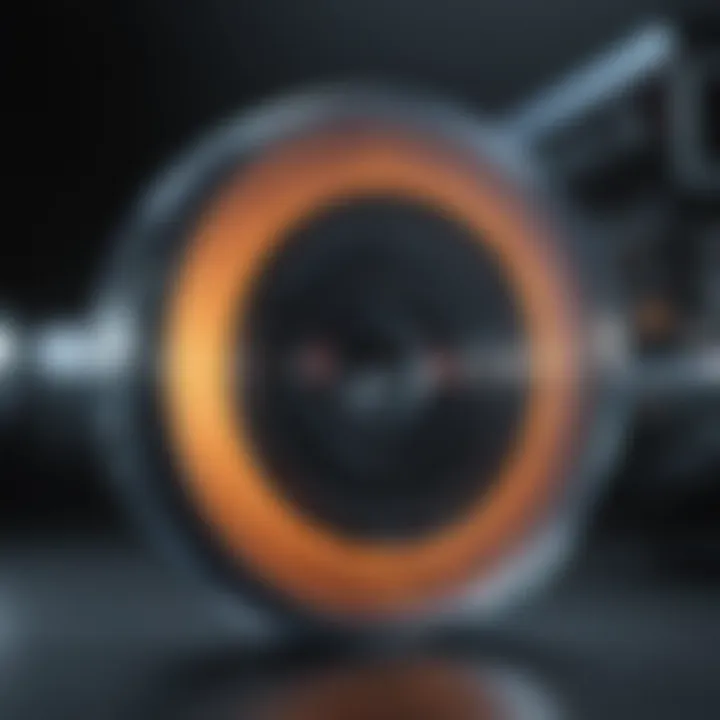
The analysis of crystalline materials is at the heart of materials science, providing detailed information about the arrangement of atoms within solids. XRD allows for the measurement of crystal structures, enabling researchers to discern the properties that arise from these atomic arrangements. A key characteristic of this technique is its precision; even subtle differences in crystal lattice structures can affect material behavior significantly. This is particularly important in the development of new materials tailored for specific functionalities, such as semiconductors and magnets.
One unique feature of XRD in this context is its ability to provide phase diagrams, which depict the stability of different phases of materials under varying conditions. However, the analysis of crystalline materials often requires high-quality samples. In cases where samples are small or impure, results can be misleading. Therefore, achieving a balance between sample quality and the depth of insight into the crystal structure is crucial in materials research.
Characterization of Nanomaterials
Characterizing nanomaterials through XRD plays a vital role in applying nanotechnology across various fields. The small dimensions of nanomaterials mean that their properties can vastly differ from those of bulk materials, making understanding their crystallographic nature essential. The key characteristic here is how XRD can be sensitive enough to detect even minor fluctuations in the lattice parameters, which can influence properties like electrical conductivity and reactivity.
A unique aspect of this characterization is the technique's ability to assess grain size using the Scherrer equation derived from broadening of diffraction peaks. This allows researchers to discern the impact of size on various materials at the nanoscale. Nevertheless, characterizing nanomaterials can pose challenges; the finite size of the samples can lead to broadening peaks and unclear data interpretations. Hence, a balance of the clarity needed against the complexity of the sample is necessary.
Chemistry
Phase Identification
Phase identification through X-ray diffraction is a critical aspect of chemical analysis. It enables scientists to determine the specific phases present in a material, which is vital for understanding its chemical composition and predicting reactions. A significant characteristic of XRD in phase identification is its non-destructive nature, allowing for the analysis of precious samples without altering their state.
One unique feature is the technique's ability to identify unknown compounds by comparing diffraction patterns with reference databases. This makes XRD a popular choice for studying complex mixtures. However, there can be limitations; overlapping peaks can complicate the identification process, particularly in samples with multiple phases. Thus, methods to deconvolve overlapping signals remain essential for achieving accurate phase identification.
Solubility and Reaction Studies
X-ray diffraction plays a critical role in solubility and reaction studies by providing insights into how the atomic structure evolves during chemical reactions. This ability to track structural changes in real-time can unveil details about reaction mechanisms that are otherwise difficult to obtain. The non-invasive nature of XRD allows for the analysis of liquid samples, providing valuable information on solubility without damaging the materials being studied.
The unique advantage of this application is its capacity for in-situ measurements, enabling a direct observation of changes occurring under varying conditions. However, accurately determining solubility can sometimes require complex interpretation of the data, particularly when multiple phases are involved in the reaction. A thorough understanding of the crystallography associated with the substances in question is essential to fully leverage XRD in this area.
Biological Research
Protein Structure Determination
X-ray diffraction has emerged as a cornerstone method in the determination of protein structures, significantly impacting biochemistry and molecular biology. The key characteristic of XRD in this application lies in its ability to yield high-resolution structures that reveal important insights into protein functionality. The process of crystallizing proteins, however, can be intricate, often requiring months of optimization.
One unique closure this technique offers is the potential to understand protein interactions, enzyme mechanisms, and drug binding sites, which are central to drug design and development. Yet, the reliance on high-quality crystals poses challenges; some proteins are notoriously difficult to crystallize, complicating this path forward in research. Thus, continued development of alternative methods that complement XRD is important for advancing this field.
Drug Crystallography
Drug crystallography through X-ray diffraction is pivotal in the pharmaceutical industry, particularly for elucidating the structures of drug compounds. Understanding the crystalline structure of a drug molecule can have profound effects on its bioavailability and efficacy. The unique ability of XRD to analyze and refine crystal structures allows for the determination of how molecular arrangement influences drug behavior within biological systems.
A notable aspect of this application is the critical need to understand polymorphism; the existence of different crystal forms can lead to variations in solubility or stability. However, the method's requirements for crystalline solid samples can sometimes limit its applicability, necessitating supplemental techniques to obtain the needed structural information. Balancing the precision of XRD with potential limitations in sample availability is a recurrent theme in drug crystallography, impacting drug development outcomes.
Thus, X-ray diffraction is a versatile and integral tool across multiple disciplines, enabling unique insights into the structures and behaviors of materials, chemicals, and biological entities. Its capacity to bridge theory with practical application continues to enhance scientific understanding and innovation in diverse fields.
Advancements in XRD Technology
In recent years, X-ray diffraction technology has tumbled down the rabbit hole of innovation. As the demand for precise and efficient techniques increases across various fields, advancements in XRD have emerged as a beacon of progress. The ability to quickly analyze samples without compromising on quality has become increasingly paramount, especially in fast-paced environments such as materials science and biotechnology. New technologies are not just enhancing existing methodologies, they're revolutionizing how researchers approach problems, leading to deeper insights and faster discoveries.
High-Throughput XRD
High-throughput XRD stands out as a notable advancement that allows researchers to manage a larger number of samples in a shorter period. This capability is crucial, particularly in fields where time is of the essence. The main characteristic of high-throughput techniques is the parallel processing of multiple samples, which offers an undeniable edge in efficiency.
Automation in Data Collection
Automation in data collection simplifies the painstaking processes traditionally associated with X-ray diffraction. By automating sample handling, data acquisition, and even initial analysis, researchers are freed from many manual tasks. This not only speeds up the workflow but also reduces the margin of error inherent in human operation. One unique feature of automated systems is their ability to operate continuously, allowing for round-the-clock data collection. Moreover, this technology enhances reproducibility, ensuring that experiments yield consistent results.
Pros:
- Significantly reduces labor costs.
- Increases throughput without sacrificing data quality.
- Minimizes human error, improving reliability.
Cons:
- Initial setup costs can be high.
- Requires specialized training to operate and maintain.
Integration with Other Techniques
The integration with other techniques marks another crucial facet of high-throughput systems. By combining XRD with complementary methods, such as scanning electron microscopy or infrared spectroscopy, researchers can gather a wealth of data from a single sample setup. This multitasking capability broadens the analysis, yielding multi-faceted insights into material properties.
The key characteristic of this integration lies in its synergistic benefits; the data from XRD can help interpret findings from other analytical perspectives, creating a holistic view of the sample under study. One unique feature is the flexibility it offers in experimental design, allowing researchers to adapt their approach based on the most pertinent results from each method.
Pros:
- Provides comprehensive insights into complex materials.
- Enables rapid troubleshooting during research phases.
- Facilitates cross-disciplinary collaborations.
Cons:
- Can complicate data analysis due to the volume of information.
- Integrating multiple systems may introduce new variables that need to be controlled.
Synchrotron Radiation and Free Electron Lasers
Another striking advancement in X-ray diffraction technology is the application of synchrotron radiation and free electron lasers. These facilities produce highly intense beams of X-rays that surpass any conventional X-ray source. This advantage stems from their ability to provide extremely bright, coherent, and tunable radiation, which is particularly advantageous for probing complex biological samples and intricate materials.
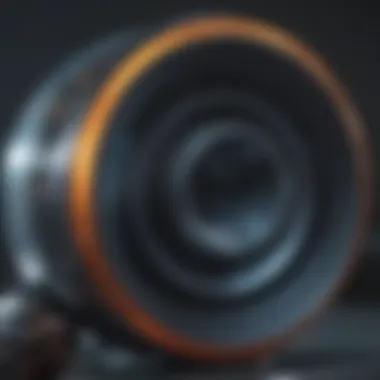
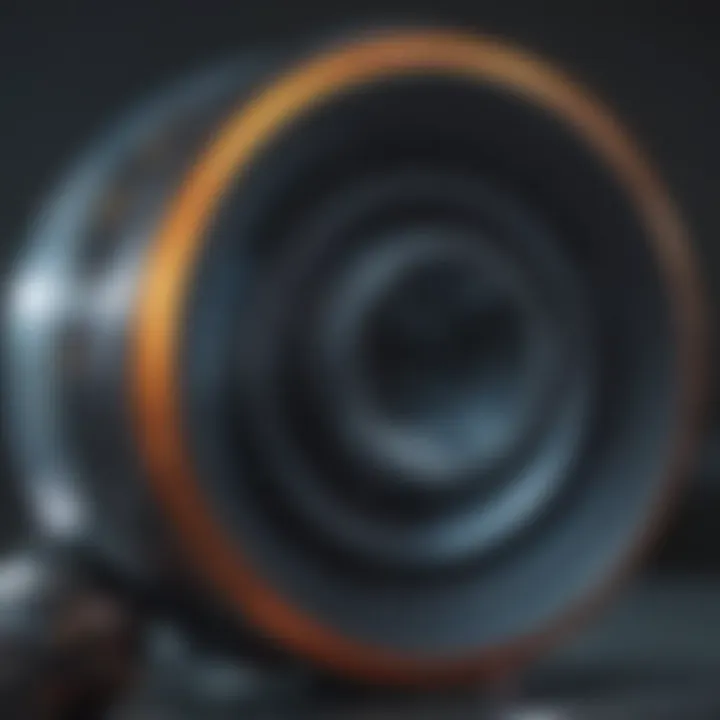
The high brightness results in faster data collection while maintaining high resolution, ultimately leading to more detailed structural insights. By harnessing synchrotron radiation, researchers can study phenomena and materials at an unprecedented level of detail, supporting advancements in fields like nanotechnology, drug development, and more.
Challenges and Limitations of X-ray Diffraction
X-ray diffraction, while a cornerstone of analytical techniques, does come with its fair share of hurdles. Understanding these challenges is essential not only for researchers looking to apply this method but also for those who aim to innovate within the field. By identifying the limitations, one can better plan for effective data collection and interpretation, ensuring that the results are valid and accurate.
Sample Size and Quality Issues
Sample size can significantly impact the results of an X-ray diffraction experiment. When the sample is too small, it might not produce a signal strong enough to yield useful data. A minuscule amount of material could lead to noise overshadowing the genuine diffraction signals, making it just like trying to spot a needle in a haystack. Moreover, quality is equally paramount. Poorly crystallized samples or those with significant defects can result in weak or inaccurate diffraction patterns. This situation necessitates stringent sample preparation protocols to enhance the chances of obtaining reliable results. Without high-quality samples, you’re essentially setting sail without a compass.
Data Interpretation Challenges
Data interpretation is arguably one of the most perplexing aspects of X-ray diffraction. The complexity lies in the intricacies of the diffraction patterns produced, which can be a maze to navigate.
Complex Peaks
Complex peaks in diffraction patterns often indicate multiple overlapping reflections. One might describe them as a crowd at a concert, where it's tough to pick out a single voice among many. These overlapping peaks can confuse the analysis process, especially when distinguishing between similar crystal structures. This becomes a stumbling block, as incorrect peak assignment can mislead conclusions about the material's structure. Understanding how to deconvolute these peaks is crucial, and it often requires the application of sophisticated software tools and a deep seeking of knowledge. In terms of their benefits, recognizing the presence of complex peaks can also lead to discovering new phases or polymorphs that may go unnoticed otherwise, making them a double-edged sword in data interpretation.
Overlapping Diffraction Patterns
Overlapping diffraction patterns present yet another layer of complexity to the analysis process. When patterns from different crystal planes overlap, it creates a cocktail of signals that can frustrate researchers. This phenomenon often results in diminished peak resolution, complicating the process of determining lattice constants or other critical parameters. Yet, there lies a unique feature here. The analysis of overlapping patterns can unveil a wealth of information about sample purity or potential mixing of phases. This nuanced understanding can lead to richer interpretations, providing insight into the material properties that would otherwise remain hidden. The key takeaway is that while overlapping patterns present challenges, they also offer unique windows into the material's structural landscape.
"Integrating challenges into the research process ultimately fosters innovation, pushing the boundaries of X-ray diffraction as a scientific tool."
Future Directions in X-ray Diffraction Research
Research into X-ray diffraction (XRD) is entering a new phase where emerging technologies and interdisciplinary approaches take center stage. The landscape of crystallography is evolving rapidly, opening doors to innovative methodologies that stand to significantly enhance the capabilities of traditional XRD. This section discusses pivotal trends shaping the future of X-ray diffraction, focusing particularly on how they foster deeper insights and broader applications across scientific fields.
Emerging Trends in Crystallography
Machine Learning in Data Analysis
Machine learning has become a cornerstone in various scientific fields, and XRD is no exception. This aspect of data analysis leverages algorithms that can learn from and interpret vast amounts of diffraction data. What makes machine learning particularly valuable in this context is its ability to spot intricate patterns and anomalies that human analysts might overlook.
One key characteristic of using machine learning in XRD data analysis is the speed with which data can be processed. Traditional methods can be time-consuming, but machine learning algorithms can rapidly crunch numbers, handling datasets that would take humans significantly longer to analyze.
However, while the advantages are evident—such as increased efficiency and enhanced accuracy—there are some hurdles to overcome. The most notable disadvantage is the need for large, high-quality datasets for training purposes. Without adequate data, the models may produce inconsistent or unreliable results, which could lead to misinterpretations in crystallographic studies.
Real-time XRD Techniques
Real-time XRD techniques have gained traction due to their potential for capturing dynamic processes as they unfold. This approach allows researchers to observe structural changes in materials under various conditions—say during phase transitions or chemical reactions—providing real-time insights that static measurements simply cannot offer.
A significant advantage of real-time XRD is the immediacy of the data. With traditional methods, researchers often must wait until the experiment concludes to analyze the results. Real-time capabilities mean that adjustments can be made on the fly if something doesn’t appear to go as planned. This responsiveness is quite advantageous in experimental settings, where conditions can be delicate and ever-changing.
On the downside, the complexity involved in setting up real-time experiments is worth noting. The instrumentation tends to be more sophisticated, which can pose challenges in terms of calibration and ensuring precision in data collection.
Interdisciplinary Applications
Integration with Quantum Computing
The confluence of X-ray diffraction and quantum computing represents an exciting frontier. The nuances of quantum mechanics could offer revolutionary improvements in simulating and understanding crystallographic systems. Quantum computing holds the promise of processing exponentially greater amounts of data, thus providing deeper insights into material properties that are currently challenging to interpret.
One compelling feature of this integration is its potential to tackle problems that require immense computational power, which classical computers struggle with. The enhanced capability for simulations could significantly streamline the exploratory phase of new materials research, promising faster development cycles and more efficient material discovery.
Nevertheless, this frontier also faces hurdles, specifically the nascent state of quantum computing technology. While the potential is considerable, practical applications in crystallography are still in their infancy, and widespread adoption may take time.
Expanding Biological Applications
The application of XRD in biological research is broadening, particularly in the realm of studying complex biological systems. Researchers are discovering more about critical elements of life, such as proteins and nucleic acids, and how their structures relate to their functions. The uniqueness of this expansion lies in its capacity to elucidate details about how biomolecules interact, which is vital for drug design and development.
A defining characteristic of these expanding biological applications is the level of detail they provide. XRD can reveal the subtle structural variations that can affect biological functions. The advantage here is substantial; understanding these structures facilitates the design of more effective therapeutic agents.
However, similar to other advanced techniques, this approach is not devoid of challenges. The requirement for highly pure samples and suitable conditions can be a limiting factor, making it less accessible for certain types of biological studies.
"The future of X-ray diffraction research is bright, with countless opportunities for innovation on the horizon. As we embrace interdisciplinary strategies, we may well be looking at the next big leap in scientific discovery."
Epilogue and Implications
The exploration of X-ray diffraction (XRD) in this article underscores its critical role in various scientific domains. As a versatile tool for investigating crystalline structures, XRD bridges gaps across materials science, chemistry, and biology. This section synthesizes the fundamental insights garnered from the preceding discussions, elucidating the nuances and future potential of XRD in research and industry.
Summary of Findings
Throughout the text, we have delved into essential principles that form the backbone of X-ray diffraction. From the historical underpinnings to the practical implications in current research, several key points emerge:
- Foundational Concepts: We discussed Bragg's Law and the reciprocal lattice concept, illuminating how these theoretical frameworks develop a deeper understanding of crystallography.
- Technological Innovations: Advancements in instrumentation, like high-throughput XRD, showcase how evolving technology enhances data collection and analysis.
- Application Spectrum: The diverse application range—from analyzing crystalline materials to investigating protein structures—illustrates XRD's versatility. Each use case lends insights that propel further scientific inquiry.
- Recognition of Challenges: Acknowledging limitations, such as sample size issues and data interpretation challenges, opens avenues for future developments.
These findings reinforce the importance of XRD as an analytical tool, revealing not just current capabilities but also the potential for expansive applications.
Significance for Future Research
Looking ahead, the domain of X-ray diffraction holds exciting prospects.
- Innovative Techniques: With ongoing integration of artificial intelligence in data analysis, researchers can anticipate streamlined data processing, leading to quicker and more accurate results. Machine learning can enhance peak identification and even assist in predicting crystal structures, which is notably complex.
- Interdisciplinary Approaches: The merging of quantum computing with crystallography could reshape how diffraction patterns are analyzed. This interdisciplinary cooperation can foster new methodologies that improve efficiencies across various applications.
- Wider Biological Implications: The acceleration of XRD in biological research, especially in drug crystallography, opens doors to novel therapeutic avenues. Understanding how substances interact at a molecular level is crucial for developing effective treatments.